James Webb Space Telescope
Welcome back, readers.
If this is the first article you have opened, I’d highly recommend reading the first part of this article. While the former was about the engineering elements
of JWST, this article is mostly focused on the physics surrounding JWST.
While discussing the
parts of JWST, we talked about the Sun Shield. For the sun shield to
successfully protect us from the heat of the Earth, Moon and Sun it should be
placed 1.5 million kilometres from Earth. The telescope is placed at a point in
space called Lagrange Point 2. It is one of the five Lagrange points.
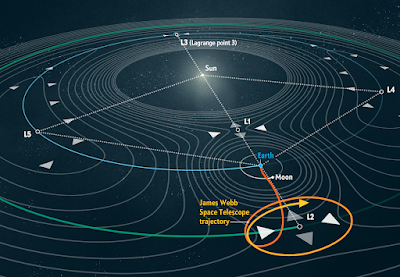 |
The five Lagrange Points |
Lagrange points are
locations in space where both the Earth and the Sun exert a gravitational pull
in the same direction. An object at this point has two gravitational forces
pulling on it to make it move in a circle. This not only allows it to orbit the
sun with a higher velocity, but it also keeps it at a fixed point relative to our
planet. The JWST orbits the sun instead of the Earth. We want the JWST to be
both further from the sun and complete a solar orbit in the same amount of time
as the Earth? To make it easier to control, the telescope would also have to
remain in the same position relative to the Earth. Here, the Lagrange point
comes into the picture.
Why Infrared?
A question that keeps
appearing, again and again, is why we have chosen infrared as our desirable
wavelength for detection?
Infrared waves have a
longer wavelength than visible light. It means it can easily penetrate dust
clouds and we can observe more far-off objects. Moreover, the light of galaxies
that are billions of light years away from us travels to us through an
ever-expanding space. This stretches the wavelength of visible light into the infrared region. Near-infrared light reveals the formation of galaxies and due
to its longer wavelength, it can pass through the dust layers that enclose the
newborn stars. Mid-infrared light peers through the cold, dusty regions where
stars form, and reveals how massive stars and black holes shape their
surroundings.
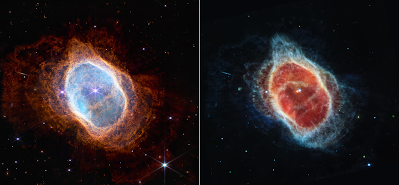 |
Southern Ring Nebulae in Near and Mid-Infrared Regions |
Moreover, various
types of celestial objects – including the planets of the solar system, stars,
nebulae, and galaxies give off energy at wavelengths in the infrared region of
the electromagnetic spectrum.
A follow-up question
that comes to mind is if we are so
adamant about looking at the Universe’s past. Why not take it to extremes and
study light in microwave regions? As far as we know the Cosmic Background
Radiation is in the Microwave region. Won’t it enable us to look further into
the past, right to the origins of the Universe?
Well, the answer is
yes and no. This means it is a little complicated…
Infrared and microwave
imaging both have their own advantages and are useful for different purposes in
astronomy. Infrared imaging is preferred over microwave imaging in many cases
because it provides higher spatial resolution and can reveal more details about the characteristics of astronomical bodies. Infrared radiation has shorter
wavelengths than microwaves, which means it can be used to study smaller
features in the Universe. In addition, infrared radiation is absorbed and
emitted by many astronomical objects, which makes it useful for studying the
temperatures and compositions of these objects. Spatial resolution is inversely
proportional to the observing wavelength. The higher the wavelength, the lesser would
be its resolution and vice-versa.
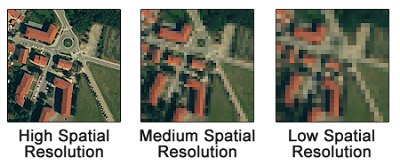 |
Different Spatial Resolutions |
Microwave imaging is preferred
in some cases because it can penetrate through clouds of gas and dust that may
obscure infrared radiation. Microwaves are also less affected by atmospheric
turbulence, which can distort images taken at other wavelengths. This makes
them useful for studying objects behind dense clouds of gas and dust, such as
the centres of the galaxies. Microwave imaging is also useful for studying the
cosmic microwave background (CMB), which is the leftover radiation from the Big
Bang.
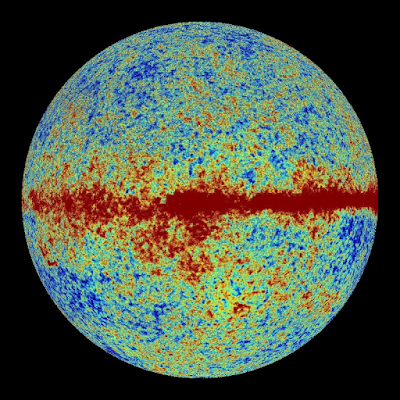 |
Cosmic Microwave Background |
In summary, both
infrared and microwave imaging have their own advantages and are used for
different purposes in astronomy. Infrared imaging is preferred due to its
higher spatial resolution and ability to reveal details about astronomical
objects, while microwave imaging is useful for studying objects behind dense
clouds of gas and dust and for studying cosmic microwave background
radiation.
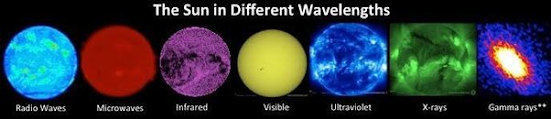 |
The Sun in different wavelengths |
The next order of business
is asking what makes Webb different from
Hubble. In what ways is Webb an upgrade or a downgrade in comparison to Hubble?
Both JWST and Hubble
are reflecting telescopes that conceptually work the same. Light reflects off a
large primary mirror onto a secondary mirror, which sends it back through a
hole in the primary mirror and into science instruments for analysis.
The basic difference
between the two telescopes is the wavelengths they work at. Hubble, which is in
Earth's orbit, is optimized for visible and ultraviolet wavelengths of light.
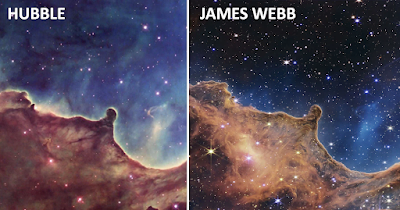 |
Karina Nebulae as seen from Hubble and Webb |
Webb essentially orbits the sun and is situated at Lagrange point 2 which is 1.5 Million Kilometres away from Earth as opposed to Hubble which is 535
Kilometers. This makes maintenance work on Hubble easier than it is for Webb.
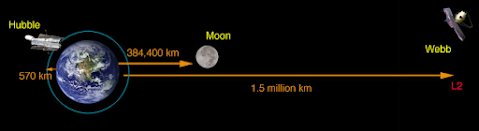 |
Distance of Hubble and Webb from Earth |
Hubble’s single mirror
is 2.4 meters (7.9 feet) wide, whereas JWST’s segmented honeycomb-shaped mirror
is 6.6 meters (21.7 feet) across. JWST has the largest mirror ever flown in
space. Tiny actuators shape each mirror to provide a single, sharp image for
the telescope’s science instruments to digest. Even though Webb’s mirror is a lot bigger in size and a hundred times more powerful, it is still 113 Kilograms
lighter than Hubble’s mirror.
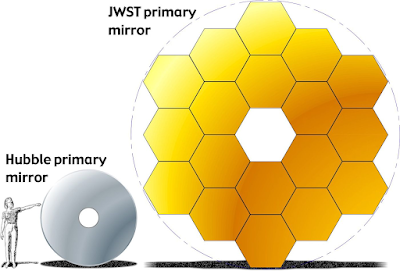 |
Hubble vs JWST Primary Mirror |
The two telescopes
also have very different cooling requirements. Hubble does not have as
sophisticated cooling needs as JWST.
How has Webb changed and challenged our
understanding of Physics?
A picture that made
big news two months back was captured by Webb. It showed multiple galaxies that
were formed way before our current understanding of Physics would permit. These
galaxies grew way too large way too soon after the big bang. News spread
everywhere. Webb has broken the Big Bang theory. So, do these pictures put our
current understanding of the Big Bang and the Standard Model into question?
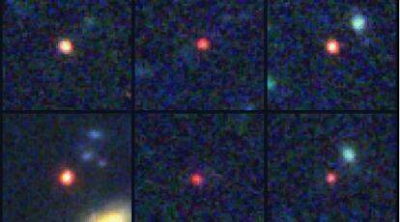 |
The oldest galaxies captured by James Webb |
As fascinating as it
might be, the answer is no. Recently, researchers took a closer look at the
data and concluded that the distant galaxies discovered by Webb are in fact in
perfect compatibility with our modern understanding of Cosmology.
As things go this
might not even be the final answer and astronomers may find galaxies at very
large distances with very large masses that puts our understanding of Physics
into question.
But, we must always
remember that in science, it’s always important to keep an open mind. For now,
we can keep the exaggerated claims to rest.
I hope you liked this
article and enjoyed reading it as much as I enjoyed writing it. Goodbye for
now.
Auf Wiedersehen!